Technical Advances in Musculoskeletal Imaging
-
Conventional data acquisition schemes
-
Acquisition of k-space data in sequential parallel lines in a 2D or 3D Cartesian grid
-
Imaging with a single RF coil, resulting in sequential acquisi tion of data
-
-
Alternative methods of sampling k-space (less subject to motion, chemical shift, and pulsation artifacts)
-
Navigation of k-space using spiral, radial, or PROPELLER trajectories
-
Oversampling of central k-space, which contains information about gross structure and image contrast
-
-
Parallel imaging (simultaneous image acquisition)
-
Imaging with an array of RF coils, resulting in the simultaneous acquisition of data over several points
-
Fewer gradient steps are necessary because spatial information is encoded by the distribution of coils as well as by spatial encoding from gradients.
-
Scan acceleration of 2 to 4 times is possible.
-
![]() |
FIGURE 1.1 ● Routine knee examination. (A) Axial T1-weighted 2D fast/turbo spin-echo image. (B) Sagittal proton density-weighted image. (C) Sagittal proton density-weighted image with chemical fat suppression. (D) Gradient-echo image.
|
![]() |
FIGURE 1.2 ● High-resolution wrist arthrography. 3D-FIESTA sequence (steady state free precession), TR 9.2 msec, TE 3.1 msec, voxel size 0.26 × 0.28 × 1.2 mm, acquisition time 3 minutes, 28 seconds.
|
![]() |
FIGURE 1.3 ● Total knee arthroplasty with synovitis (arrows). Acquired at 1.5 T. Use of an RBW of 83.3 kHz results in limited metallic susceptibility artifact.
|
![]() |
FIGURE 1.4 ● Magic angle effect (arrows). (A, B) Magic angle is seen in a shoulder examination using a short TE (A) and long TE (B). Magic angle in an ankle examination without (C) and with (D) FatSat.
|
accelerated scan (SNR ASSET) are illustrated in the following equation:
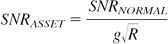
![]() |
FIGURE 1.5 ● (A) PROPELLER fast spin-echo with a 480 × 480 matrix. (B) Conventional 2D fast spin-echo with 480 × 384 matrix. Scan times were identical. Note an apparent SNR gain in (A) as well as better delineation of cartilage and menisci.
|
![]() |
FIGURE 1.6 ● Example of parallel imaging: ASSET/SENSE with acceleration × 2. Half of the raw data are acquired, resulting in half the scan time. SMASH/GRAPPA techniques work in the k-space domain instead of the image domain.
|
![]() |
FIGURE 1.7 ● SENSE/ASSET acceleration × 2 with an eight-channel wrist array coil. Axial 2D fast spin-echo (TR 700, TE 16, ETL 2, slice thickness 2 mm, FOV 10 cm, 384 × 320 matrix, 15 slices). With ASSEST × 2 (A), scan time was 58 seconds. Without ASSET (B), scan time was 1 minute 54 seconds. The SNR reduction with ASSET is minimized due to the optimal coil g-factor.
|
-
A series of spin echoes fill lines of k-space, reducing scan time.
-
The number of echoes in the series is the echo train length (ETL), usually 3 to 16.
-
The time between the echoes in the echo train is the echo train space (ETS), usually 4 to 10 msec.
-
The full time to collect the echoes is the sum of the ETL and ETS between each echo.
-
Signal loss from T2 relaxation occurs progressively during the echo train. Therefore, tissues with a short T2 relaxation do not contribute data to the late echoes that encode for spatial resolution, and image blurring results.
-
Since the T2 relaxation time of most musculoskeletal tissues is relatively short, a short echo space minimizes image blur.
-
Decreasing echo space is best accomplished by increasing the receive bandwidth (RBW).
techniques in the early 1990s3 revolutionized MR imaging by allowing the collection of spin-echo data in less time than any of the then currently available gradient-echo or spin-echo sequences. In conventional spin-echo imaging, each echo generates one image. In fast spin-echo imaging, a series of spin echoes, called the echo train, fill lines of k-space, thereby reducing scan time. The series of spin echoes are generated by the use of 180-degree refocusing RF pulses at regular intervals. After each 180-degree pulse, an echo signal is collected that fills a different line of k-space (Fig. 1.8). The images are then reconstructed from the data collected over many echo times (TEs). Scan times are reduced by a factor equal to the number of echoes, the ETL. ETLs vary from 3 to 16. The time between the echoes in the echo train is called the echo train space (ETS) and is generally 4 to 10 msec.
![]() |
FIGURE 1.8 ● Fast or turbo spin-echo. After each 180-degree refocusing pulse, an echo signal is collected and fills a different k-space line. This accelerates data collection by a factor equal to the ETL.
|
the early echoes of the echo train are seen in structures with a T2 of 10 msec. For tissues with a long T2 (100 msec for instance), all the echoes in the train are seen. The early echoes in the echo train contribute information from central k-space and improve SNR and produce image contrast. The later echoes in the train, with information from peripheral k-space, improve spatial resolution. Consequently, blurring occurs if a long echo train or echo spacing is used for tissues with a short T2 relaxation time, since the raw data from the late echoes that encode high-spatial-frequency details are missing. To minimize blurring when imaging tissues with a short T2 relaxation time, shortening of the ETL and/or the ETS may be required.
![]() |
FIGURE 1.9 ● Short T2 relaxation times and echo train in fast or turbo spin-echo imaging. If echo spacing is too long, the high spatial resolution is missing from the late echoes, resulting in increased blurring.
|
![]() |
FIGURE 1.10 ● Effect of RBW on fast spin-echo. Note (A) the chemical-shift effect (arrows) on the bone-cartilage interface and (B) the increased blurring due to the longer echo spacing with ± 7 kHz.
|
TABLE 1.1 ● Effects of Increasing Receiver Bandwidth and Frequency Matrix on Fast Spin-Echo Performance
|
|||||||||||||||
---|---|---|---|---|---|---|---|---|---|---|---|---|---|---|---|
|
-
STIR imaging
-
Based on the differences in longitudinal relaxation of fat vs. water
-
Advantage: it is relatively unaffected by inhomogeneities in the magnetic field and radiofrequency field
-
Disadvantages: long scan times, relatively fixed TRs and TEs, diminished image contrast, and insensitivity to gadolinium due to inadequate T1 weighting
-
-
ChemSat or FatSat imaging
-
Based on the differences in precessional frequency of protons in fat vs. water
-
Uses a spectrally selective pulse to image the frequency shift between fat and water protons
-
Advantages: flexibility in TR and TE, sensitivity to gadolinium, ability to be used with fast gradient-echo techniques
-
Disadvantage: sensitivity to inhomogeneity in the magnetic field and in the RF power distribution, resulting in uneven fat suppression
-
-
Dixon imaging
-
Based on the differences in precessional frequency of protons in fat vs. water
-
Uses a nonspectrally selective pulse with TEs that capture phase difference between fat and water protons
-
Advantages: flexibility in use of TR, TE, and pulse sequences and its relative insensitivity to inhomogeneity in RF power distribution
-
Disadvantages: sensitivity to magnetic field inhomogeneity and potential image blurring due to long echo spacing
-
![]() |
FIGURE 1.11 ● The formula for the chemical shift effect, expressed in number of shifted pixels, is:
![]() For example, with an RBW of ± 16 kHz, the chemical shift is 256 × 220 divided by 32,000, or 1.76 pixel.
|
![]() |
FIGURE 1.12 ● Signal versus noise as a function of the RBW. With ± 32 kHz, the amount of noise that the coil “sees” has doubled, whereas the signal intensity remains the same (red area under curve unchanged).
|
-
Flexibility in image contrast without the TR/TE restrictions characteristic of STIR sequences (Fig. 1.14)
-
Sensitivity to gadolinium
-
Ability to be used with fast gradient-echo techniques with very short TR and TE
fat magnetization vectors. At 1.5 T, fat precesses at 220 MHz slower than water. At this difference in precessional rate, the time for the phase difference to complete a rotation of 360 degrees is 4.6 msec. Therefore, the TE for in-phase imaging is 4.6 msec or a multiple of 4.6 msec. At this rate, the fat and water vectors will be maximally out of phase, at 180 degrees to each other, at 2.3 msec. Consequently, the TE for out-of-phase imaging is 2.3 msec or a multiple of 2.3 msec. The fat and water in-phase and fat and water out-of-phase images can then be added and subtracted to produce water-only or fat-only fat-suppressed images.
![]() |
FIGURE 1.13 ● STIR imaging with a large FOV improves image quality in challenging areas such as (A) the brachial plexus and (B) the spine. (C) T2-weighted image for reference. In such applications, chemical fat saturation may show uneven fat suppression.
|
![]() |
FIGURE 1.14 ● Fast spin-echo proton density-weighted images without (A) and with (B) FatSat. On the FatSat image, fluid and cartilage appear brighter relative to the suppressed fat signal of bone marrow and subcutaneous fat.
|
TABLE 1.2 ● Comparison of STIR and FatSat Techniques for Fat Suppression, Part I
|
||||||||||||
---|---|---|---|---|---|---|---|---|---|---|---|---|
|
TABLE 1.3 ● Comparison of STIR and FatSat Techniques for Fat Suppression, Part II
|
||||||||||||
---|---|---|---|---|---|---|---|---|---|---|---|---|
|
![]() |
FIGURE 1.15 ● Fat-water separation technique (IDEAL). Images are acquired in three scans with slightly different echo times (and therefore different phase characteristics). The images then undergo phase analysis reconstruction, resulting in images of pure water and fat, as well as a variety of other combinations (such as combined water and fat, in-phase and out-of-phase).
|
-
Metal causes local magnetic field distortion that results in misregistration artifact and intravoxel dephasing.
-
Methods for diminishing metal artifact include:
-
Positioning the long axis of a metallic prosthesis parallel to the axis of the magnetic field if possible
-
Aligning the frequency-encoding gradient along the axis of a metallic prosthesis
-
Increasing the frequency-encoding gradient strength
-
Increasing the matrix in the frequency-encoding direction
-
Increasing the RBW
-
Decreasing the FOV
-
Using fast spin-echo techniques with a long echo train
-
![]() |
FIGURE 1.16 ● Comparison of conventional ChemSat (A) with fat-water separation (IDEAL) (B). Note the improved fat suppression in bone marrow, especially in peripheral areas (arrows).
|
perpendicular to the field. The manipulation of several other MR parameters can help to reduce metal artifacts.6 Due to misregistration, metal artifacts are usually greatest in the frequency-encoding direction and are inversely proportional to the frequency-encoding gradient strength. Aligning the frequency-encoding direction along the axis of the metallic implant, increasing the frequency-encoding gradient strength, and increasing the matrix in the frequency-encoding direction all help to decrease the artifact. If the most important images for diagnosis are in the coronal and sagittal planes, frequency-encoding performed in the anterior-posterior direction and phase-encoding in the superior-inferior direction will improve image quality. Increasing the RBW and decreasing the FOV are also critical in minimizing metal artifact (see Fig. 1.17B). Sequence selection is also important. Fast spin-echo images with a long ETL result in less artifact than conventional spin-echo images and much less artifact than gradient-echo sequences. Scans should not be performed with FatSat, since this will accentuate artifacts. Shorter TE sequences result in less artifact. However, TR is not an important variable.
![]() |
FIGURE 1.17 ● Metal artifact reduction. Coronal fast spin-echo images (TR 3000, TE 44) (A) without a metal artifact reduction technique (ETL 8, RBW 19.2) and (B) with a metal artifact reduction technique including increased RBW and long ETL (ETL 16; RBW 83.3).
|
-
Cartilage T2 mapping
-
Uses multi-echo sequence with post-processing and color map
-
Used for assessment of collagen degeneration
-
Cartilage T2 signal variability may come from normal variation in collagen concentration and collagen fiber orientation
-
-
Cartilage T1 rho
-
Uses multiple 3D gradient-echo scans with various flip angles
-
Used for assessment of glycosaminoglycan (GAG) and proteoglycan content
-
Decreased GAG content results in elevated T1 prolongation
-
-
Cartilage dGEMRIC mapping
-
Uses intravenous injection of gadolinium, followed by 10 minutes of exercise, and an 80-minute wait period before imaging
-
Uses T1 sequences with fast inversion recovery pulse
-
Is used for assessment of GAG content
-
Decreased GAG content results in increased contrast uptake and decreased T1 value
-
![]() |
FIGURE 1.18 ● Spin-echo, 8 echoes (10 to 80 msec). Note the signal attenuation in the patellar cartilage. This type of sequence is used to produce cartilage T2 color maps.
|
![]() |
FIGURE 1.19 ● Color map (A) showing T2 values from 20 msec in red to 75 msec in blue, with calculated T2 values from the region of interest (ROI). ROI #1 green curve (B) shows the 8-echo signal pattern, and the red curve shows the calculated monoexponential fit.
|
![]() |
FIGURE 1.20 ● Clinical examples of T2 mapping at 3 T. Color map showing T2 values from 25 msec in red to 75 msec in blue. (A, B) Osteoarthritis (arrow) is not visible on the conventional T2 image (A). (C, D) Autologous osteochondral implant (mosaicplasty) of the medial femoral condyle with mild prolongation of T2 values. (Courtesy H.G. Potter, HSS, NY)
|
![]() |
FIGURE 1.21 ● dGEMRIC T1 maps at 1 T. (A) Asymptomatic volunteer (in-plane resolution 450 μ × 450 μ). (B) Patient post-autologous chondrocyte transplantation (in-plane resolution 570 μ × 570 μ). The graft is indicated with an arrow.
|
-
Diffusion-weighted imaging (DWI)
-
Based on proton mobility or diffusivity
-
Potential application for evaluation of cartilage degeneration
-
-
Diffusion tensor imaging (DTI)
-
Based on directional proton mobility along fiber tracts
-
Potential applications for evaluation of neuromuscular degenerative diseases, muscle injury, and peripheral nerve pathology
-
![]() |
FIGURE 1.22 ● Example of a totally isotropic 3D sequence, 3D-VIPR. Raw data are acquired in a projection-reconstruction mode (not like 2D and 3D Fourier). Reformations (A –C) from a unique 3D data set of 0.4 × 0.4 × 0.4 reconstructed voxels, acquired in 5 minutes 1 second. The sequence also provides an intrinsic fat suppression and a T1/T1 image contrast (steady-state free precession). (Work in progress, developed by University of Wisconsin, Madison)
|
also known as the b-value. The b-value can be increased by increasing the amplitude of the gradients, the duration of the gradient applications, and the time interval between the two gradient pulses. In the images produced, the apparent diffusion coefficients (ADCs) of each voxel can be measured.
![]() |
FIGURE 1.23 ● Principle of diffusion imaging. (A, B) Biologic tissue shows extracellular fluid random motion (e.g., diffusion) affected by tissue condition. (C, D) By adding specific diffusion sensitization gradients, spins experiencing diffusion result in an attenuated signal due to a net phase shift, unlike stationary spins with no attenuation.
|
to as anisotropic diffusion. Fiber tracts or myelin sheaths, for example, may limit the direction in which molecules can move. Diffusion tensor imaging (DTI) is a form of DWI that images the directional information of structures such as white matter tracts (Fig. 1.24). Recent advances allow the diffusion data to be processed in color 3D to highlight the trajectories of continuous anisotropy, which are closely correlated with the physical fiber patterns. These direction-encoded color maps, DTI fiber tractography, are depictions of neuronal networks.
![]() |
FIGURE 1.24 ● Diffusion anisotropy. (A) Free water shows non-oriented diffusion behavior. (B) Posterior view of motor innervation. White matter tracts or muscle fibers show diffusion properties that reflect the orientation or path of the tracts or fibers. In the medulla oblongata corticospinal axons cross the midline in the pyramidal decussation. Ventral roots (motor) reach skeletal muscles through the peripheral nerves.
|
![]() |
FIGURE 1.25 ● Examples of diffusion tensor MRI in calf muscle. (A) Blue indicates diffusion along the S-I axis. (B) Fractional anisotropy shows the degree of anisotropy regardless of direction. Control image (C) and fiber-tracking display using two different rendering techniques (D, E).
|
![]() |
FIGURE 1.26 ● Hip examination, coronal fast spin-echo proton density-weighted image with FatSat (TR 3100, TE 41). (A) Images acquired with a four-channel cardiac array coil. (B) Images acquired with a body coil. The signal-to-noise improvement with the array coil is at least 100%.
|
![]() |
FIGURE 1.27 ● Bilateral temporomandibular joint study, with two circular 3-inch coils. Note the strong signal attenuation from outside to inside. As a rule, the optimal depth zone for a circular coil is equal to its radius-that is, about 1.5 inches (4 cm) from the coil inner surface.
|
![]() |
FIGURE 1.28 ● Phased-array knee coil. (A) Split-top design. (B) Coil tapered to the knee shape to maximize SNR and patient comfort. Coil is made of an RF transmit birdcage (C) and eight longitudinal RF loops for reception (D).
|
![]() |
FIGURE 1.29 ● Examples of dedicated musculo-skeletal RF coils. (A) Three-channel shoulder (the shoulder array coil is available in four- and eight-channel designs) array coil; note the underarm RF loop. (B) Four-channel (the eight-channel wrist array coil is also used in the neutral wrist position) wrist array coil. This coil can also be used in the horizontal position, closer to the magnet isocenter, with the patient prone and with the arm extended above the head (the “Superman” position). This less-comfortable position is sometimes required when the system does not deliver good image quality with widely off-centered coil positions.
|
![]() |
FIGURE 1.30 ● Image quality improvement with an eight-channel knee array coil (A) compared to a four-channel extremity coil (B). Note the increased SNR in the patellofemoral cartilage area.
|
![]() |
FIGURE 1.31 ● 3-T imaging of the wrist. Coronal proton density-weighted image (A) and axial proton density (B) and proton density fat-suppressed (C) images of the wrist.
|
depth, and then increases centrally. This variation in B1 may result in image edge and center brightening. For most musculoskeletal applications, however, the potential benefits with higher-resolution imaging and improved fat suppression are substantial.11
![]() |
FIGURE 1.32 ● Sagittal proton density-weighted images of the knee acquired with identical parameters at 3 T and 1.5 T. Note the improved SNR of the 3 T image (A) versus the 1.5-T image (B).
|